Plasma Waves Studied for New Electronics
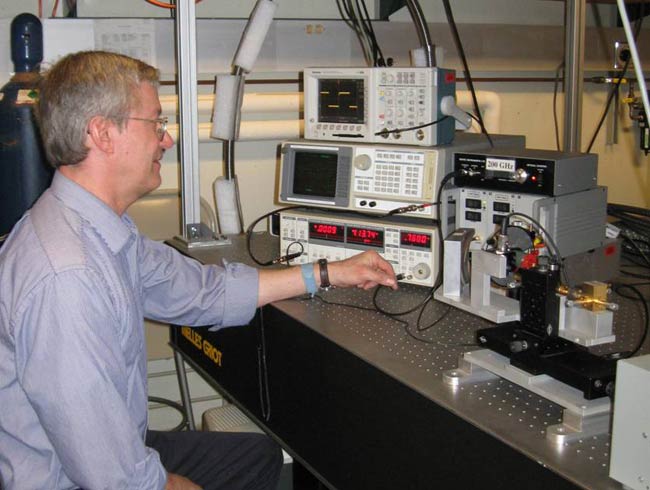
This Behind the Scenes article was provided to LiveScience in partnership with the National Science Foundation.
Who says you can’t teach an old dog new tricks?
After nearly 25 years as an electrical engineer in semiconductor development and manufacturing, I headed back to school as an NSF Integrative Graduate Education and Research Traineeship (IGERT) fellow.
The focus of my research involves terahertz radiation, about which I knew very little when I first arrived on campus. Up until recently, the terahertz portion of the electromagnetic spectrum has mainly been of value to studies of atmospheric and astronomic phenomena, such as mapping the changes in the Earth’s ozone layer and peering into the origins of the universe. Now, though, applications are exploding.
Terahertz radiation is part of the electromagnetic spectrum, just like sunlight, radio waves, X-rays and gamma rays. Terahertz frequencies are between 0.3 and 30 trillion cycles per second, leading to some very interesting properties.
For one, terahertz radiation tends to pass through many common things like paper, cardboard, leather and fabric, to more exotic materials, like the sprayed-on foam insulation of the space shuttle, and it’s reflected by metals and many ceramics. This makes it really good at finding hidden objects, such as weapons hidden under clothing at the airport or defects in the adhesion of that shuttle foam (which could lead to failure during launch).
Since terahertz radiation wavelengths are in the range of 1 to 0.01 millimeters, good image resolution is possible, and yes, in case you’re wondering, this brings up privacy issues, which are a hot debate at the moment.
Get the world’s most fascinating discoveries delivered straight to your inbox.
While we’re on the subject of imaging, since the radiation frequencies coincide with many vibration modes for molecules, not only can you see that something is hidden, you can figure out what it’s made of. For example, you can see whether the powder contained in an envelope is talc or methamphetamine, or whether an improvised explosive device is made from dynamite or more powerful plastic explosives.
However, terahertz radiation is nearly completely absorbed by water, including moisture in the air, so the standoff distance — how far away we can see those threats — is limited to about 100 meters (328 feet).
For some applications this limited transmission distance is an advantage, such as for secure tactical communications where long signal ranges increase the likelihood of interception. Similarly, it is the difference in cellular water content, and hence terahertz absorption, which allows identification of cancerous tissue in medical applications.
Although deep tissue scans, like X-ray scans, are not possible, analysis can be performed on excised samples. And unlike X-rays, terahertz radiation is non-ionizing, so repeated exposure does not pose a health risk.
With all of this potential, why aren’t terahertz applications all over the place? One reason is the available power of terahertz sources. Compared to a typical mainstream FM radio station, running at 100 kilowatts, terahertz-source power tops out in the range of a few hundred milliwatts.
The difficulty in coming up with high-power terahertz sources lies in the frequency range: it's "too fast" for electronic sources and "too slow" for optical ones. The low power of available sources puts a significant burden on the other side of the equation, the sensitivity of the terahertz detector. While atmospheric and astronomic applications have long relied on running detectors at cryogenic temperatures and converting terahertz signals to lower frequencies (downconverting), the lion’s share of earth-bound applications really have to operate at room temperature and, oh, by the way, they need to be fairly small and fairly cheap.
Certainly available detectors exist today, namely pyroelectric devices, where heat energy is converted to an electrical signal due to a change in material polarization, and compound semiconductor Schottky diodes, in which non-linear operating characteristics turn the received signal into a DC voltage.
Both of these devices have their limitations, though: pyro-electric devices are on the slow side and C.S. Schottky devices are difficult to integrate with complex integrated circuits. If only there was a way to make conventional transistors operate at terahertz frequencies...
Enter plasma-wave electronics, the topic of my research. The speed of conventional, transit-time silicon Field Effect Transistor (FET) operation is limited to roughly 0.5 to 0.8 terahertz (even with nanoscale devices).
Electron plasma waves are localized, time-varying perturbations in the electron concentrations in the FET channel; think of them as waves on a pond when you toss in a pebble.
First proposed in 1973 by Michael Dyakonov and my thesis advisor Michael Shur, plasma-waves are expected to allow silicon FETs to operate as high as 10 terahertz! In our lab, we’ve demonstrated detection from 0.2 to 1.6 terahertz with these devices, and terahertz detectors based on silicon FETs will be easy to integrate with complex image processing circuits.
In the process of our research, we’ve found a way to apply terahertz radiation to testing large scale integrated circuits, memory chips and microprocessors for defects and traits that can hamper performance.
However, the most rewarding aspect of my research, by far, is the collaboration with my peers and mentors, and our research group has a strong international component; I’ve grown from my experiences here tremendously. I received my Ph.D. in electrical engineering this past May, and am continuing my research as a post-doctoral research associate. On top of all of this, last semester I had the opportunity to teach a sophomore year physics class as an adjunct professor. Quite a few new tricks for this old dog!
- All About Light
- Video - The Next Step in Revolutionary Electronics
- 10 Technologies That Will Transform Your Life
Editor's Note: This research was supported by the National Science Foundation (NSF), the federal agency charged with funding basic research and education across all fields of science and engineering. See the Behind the Scenes Archive.