
'Synthetic' Leaves: The Energy Plants of the Future? (Kavli Roundtable)
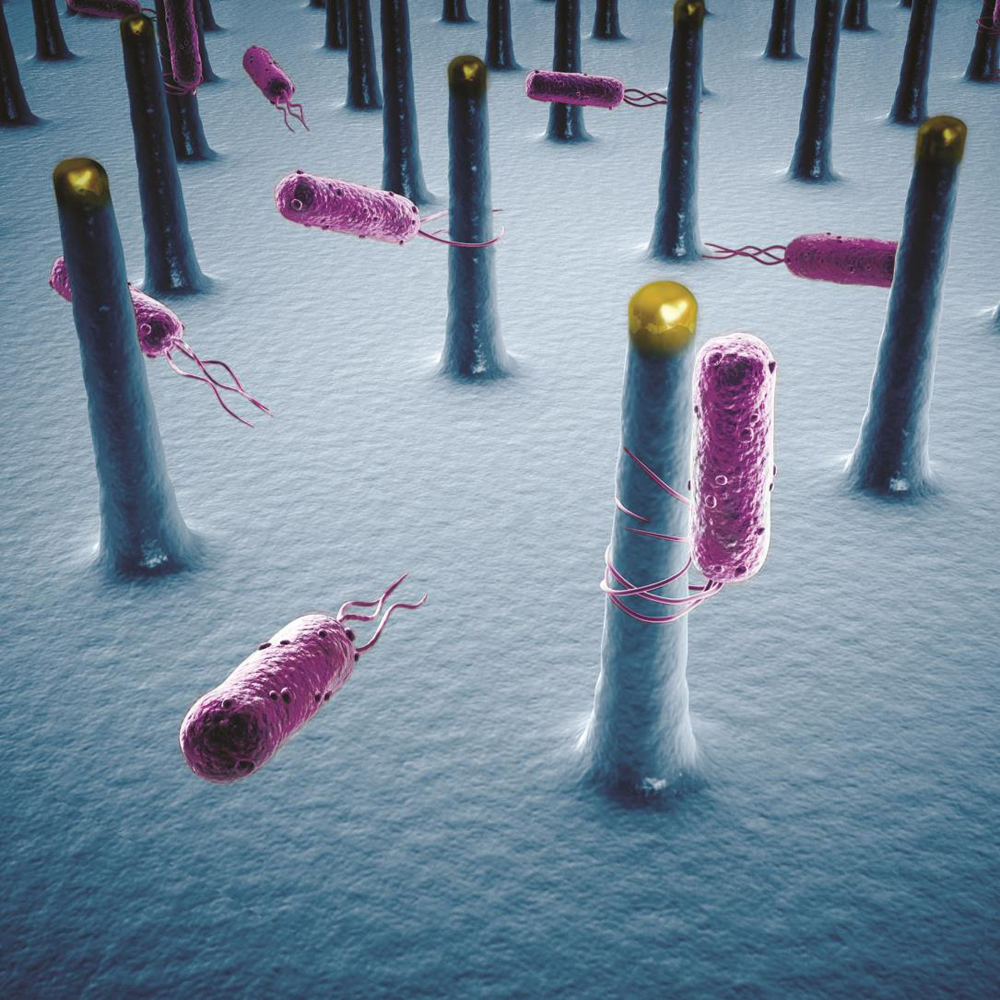
Alan Brown, writer and blogger for The Kavli Foundation contributed this article to Live Science's Expert Voices: Op-Ed & Insights.
Imagine using plants to grow the natural gas that heats homes and the gasoline that powers cars. People could store it this form of solar energy in cars' fuel tanks, distribute it through pipelines, and buy it in gas stations. And everyone could use it without adding a single molecule of the greenhouse gas carbon dioxide (CO2) to the atmosphere.
Green plants and some bacteria basically do this every day, through photosynthesis, turning water and carbon dioxide into sugar. Sugar is an organic fuel that stores the sun's energy for plants to use at night or when they awake leafless in the spring. But suppose engineers could tweak this natural process to produce natural gas or gasoline?
Advances in nanoscience are rapidly bringing that vision closer to reality. In a recent paper published in Nano Letters, Peidong Yang, co-director of the Kavli Energy NanoSciences Institute and professor of chemistry at the University of California, Berkeley, led a team that achieved synthetic photosynthesis by combining nanoscale semiconductors and genetically modified bacteria.
By marrying nanoscience and biology, Yang and his colleagues created a biologically inspired, but completely artificial, system that converts the sun's rays into fuel and chemicals. The system uses long, nanoscale filaments to turn sunlight into electrons, which bacteria use to convert carbon dioxide and water into butanol fuel and more complex molecules such as acetate, a chemical building block, and amorphadiene, which is used to make antimalarial drugs.
This past August, Yang's team used a similar approach to make methane, the most important component of natural gas. It used nanowires to split water into oxygen and hydrogen, and hydrogen-loving bacteria to turn CO2 into methane.
The Kavli Foundation invited three leading researchers to discuss this promising technology, the roadblocks that remain before it becomes commonplace, and how science might learn from nature's genius.
The participants were:
Peidong Yang, co-director of the Kavli Energy NanoScience Institute at Berkeley National Laboratory and a professor of chemistry at the University of California, Berkeley. Yang serves as director of the California Research Alliance by BASF and was a founding member of the U.S. Department of Energy (DOE) Joint Center for Artificial Photosynthesis (JCAP).
Thomas Moore is a professor of chemistry and biochemistry and past director of the Center for Bioenergy & Photosynthesis at Arizona State University. He is a past president of the American Society for Photobiology, and a team leader at the DOE Center for Bio-Inspired Solar Fuel Production.
Ted Sargent is a professor of electrical and computer engineering at the University of Toronto where he is chair for nanotechnology and vice-dean for research for the Faculty of Applied Science and Engineering. He is also the founder of two nanotechnology companies: InVisage Technologies and Xagenic.
The following is an edited transcript of their roundtable discussion. The participants have had the opportunity to amend or edit their remarks.
TKF: Solar cells do a good job of converting sunlight into electricity. Converting light into fuel seems far more complicated. Why go through the bother?
Thomas Moore: That's a good question. In order to create sustainable, solar-driven societies, we need a way to store solar energy. With solar cells, we can make electricity efficiently, but we cannot conveniently store that electricity to use when it is cloudy — or at night. If we want to stockpile large quantities of energy, we have to store it as chemical energy, the way it is locked up in coal, oil, natural gas, hydrogen and biomass.
Peidong Yang: I agree. Perhaps, one day, researchers will come up with an effective battery to store photoelectric energy produced by solar cells. But photosynthesis can solve the energy conversion and storage problem in one step. It converts and stores solar energy in the chemical bonds of organic molecules.
Ted Sargent: Much of the globe's power infrastructure — from automobiles, trucks and planes to gas-fired electrical generators — is built upon carbon-based fossil fuels. So creating a new technology that can generate liquid fuels that can use this infrastructure is a very powerful competitive advantage for a renewable energy technology.
Also, our energy needs change with the seasons. Here in Canada, heating drives up energy use in winter. Maybe we could build a battery to store enough energy to heat our homes overnight, but the greater long-term challenge is to store energy we capture in the summer and use it to heat our nation of 35 million people in the winter.
The remarkable energy density of fossil fuels, all of which store energy created by ancient photosynthesis, make this possible. So while converting sunlight to fuels will always have a greater energy cost than making electricity, liquid fuels have a notably higher value because they can meet seasonal gaps between the supply and demand of renewables.
And, finally, synthetic photosynthesis is a carbon-neutral solution, because we take one CO2 molecule out of the atmosphere for every CO2 molecule that we return during combustion.
T.M.: As Ted implied, the driver behind this is that the global carbon cycle is completely out of control. Burning fossil fuels is putting CO2 in the atmosphere much faster than photosynthesis can take it out. A system that pulls every carbon [atom] that we burn out of the air and converts it into fuel is truly carbon neutral.
[Atmospheric] CO2 levels surpassed 400 parts per million this year. If they reach 500 or 600 parts per million, the environmental impact is going to be severe. We will need some form of carbon capture and storage. This leads right into Peidong's system, because it could remove copious amounts of CO2 from the atmosphere, use some for fuel, and make carbon rocks out of the excess. In that way, it could reduce atmospheric CO2 to pre-industrial levels.
TKF: Professor Yang, you created a photosynthesis system that is half synthetic and half natural. What gave you the idea?
P.Y.: The story starts more than 10 years ago, when Berkeley designed a fully integrated solar-to-fuel generator. We tried to mimic what goes on in natural photosynthesis.
We used semiconductors to capture solar energy and generate current. We used the current to energize two catalysts — materials that speed up chemical reactions without actually taking part in them. One catalyst reduced, or added electrons to, CO2, and the second oxidized [took electrons from] water to produce oxygen, which is what happens in natural photosynthesis. The synthetic CO2 catalysts were the problem, because they were simply not very efficient.
So about five years ago, we decided to try using nature to play the role of those CO2 catalysts. Some bacteria, such as Sporomusa ovata (S. ovata) actually have the capability to reduce CO2 with very, very high selectivity, meaning they deliver electrons to CO2 to make one specific organic molecule and nothing else.
In our system, we still use inorganic materials to capture sunlight and generate electrons. But we send the electrons to the S. ovata, which use them to turn CO2 into acetate, a more complex molecule. Then we use a second bacterium, Escherichia coli (E. coli) to turn acetate into more complex chemicals.
TKF: Do you think this type of hybrid system — a combination of synthetic light converters and natural catalysts — is the way of the future?
P.Y.: Honestly, I'm not so sure this is the best way to create an artificial photosynthetic system.
We're good at generating electrons from light efficiently, but chemical synthesis always limited our systems in the past. One purpose of this experiment was to show we could integrate bacterial catalysts with semiconductor technology. This lets us understand and optimize a truly synthetic photosynthesis system.
Ultimately, we would like to take what we learn and develop a synthetic catalyst with performance similar to the bacteria. That would let us put together a much more robust, fully integrated solar-to-fuel generator. Meanwhile, our current approach represents an intermediate step that lets us learn about artificial photosynthesis in new ways.
T.S.: Peidong's right to put the focus on exactly this question: What can biology teach us about making fuels? His model system makes it possible to explore some really important physics and chemistry. This is not about mimicking nature directly or literally. Instead, it is about learning nature's guidelines, its rules on how to make a compellingly efficient and selective catalyst, and then using these insights to create better-engineered solutions.
TKF: Is there a way to create the type of synthetic catalysts Professor Yang envisions?
T.S.: Nature has figured out efficient CO2-to-liquid-fuel catalysts. We have not yet managed to do that. In particular, as Peidong noted, we need high selectivity to make the product we want without undesired side products. We also need catalysts that convert chemicals quickly, and without making us pay an energy penalty for their high throughput. Finally, nature builds catalysts using abundant materials. On all these fronts, nature has us beat. But it is also exciting, because nature proves it's possible. This is a problem that has been solved before.
T.M.: Those are extremely good points. Nature's catalysts are remarkable for a number of reasons. They self-assemble, and nature repairs any damage to them. They always use abundant materials because nature does not mess with anything that is rare or expensive. They always work at ambient temperatures.
As Ted said, nature's catalysts do not require a lot of excess energy. When chemists want a chemical reaction to go faster, we heat it up or apply more voltage. Nature did not have either option, so it had to solve the problem by finding a low-energy pathway.
Again, as Ted and Peidong mentioned, selectivity is hugely important. Our industrial society expends lots of energy separating desired chemicals from all the other junk we make along the way. Nature makes what it wants, and it's nearly always already pure.
Nature proves it's possible, but we are still a ways away from having nature's catalytic prowess. But Peidong's work establishes that technology and nature can work together.
TKF: Let me return to something Professor Yang mentioned earlier. Your system is making a chemical called acetate. Why is that important?
P.Y.: CO2 has one carbon atom, so it is relatively easy to make a chemical with one carbon atom from CO2. But it is much more desirable — and difficult — to create a chemical with more than one carbon atom. Acetate has two carbons, and our hybrid system proves that we can create a molecule like this.
While acetate is not necessarily our most desired end product, it is a common building block in biosynthesis. In our study, my Berkeley collaborator, Michelle Chang, genetically modified E. coli to turn acetate into more interesting chemicals, such as butanol fuel, biodegradable polymers and drug precursors.
If we could design a synthetic catalyst that did this sort of carbon-carbon coupling at room temperatures and pressures, that would be fantastic. However, we do not know how to do that yet.
T.M.: I think that Peidong is being a little modest about making acetates. I mean, if you go from CO2 to acetate, all the heavy lifting is already done. You've produced a carbon-carbon bond.
TKF: Why is that so important?
T.M.: Because the two-carbon unit is the fundamental feedstock for a whole mess of different metabolic pathways. For example, when our body metabolizes the fatty acids we eat, it chops them up into two-carbon units. From those two-carbon units, it makes everything it needs. So carbon-carbon units are very important in metabolism, much more common than single carbon units.
TKF: So acetate is a good building block?
T.M.: Yes, and there are organisms that would love to build with it. Plus, as we learn more, we can use that knowledge to create synthetic catalysts to make butanol, gasoline, longer chain hydrocarbons — it is all thermodynamically possible once you get acetate. So it is a big deal.
T.S.: It is, especially for fuels.
TKF: Professor Yang, one of the unusual aspects of your hybrid system is that it uses nanowires to convert light into electrons. Why use nanowires instead of more conventional solar panels?
P.Y.: That relates to the one key requirement of the original design: We want transfer electrons from our semiconductors to our S. ovata bacteria, which act as our CO2 catalysts. To do that, we want the highest possible surface area, so that we put more bacteria in contact with the semiconductors and reduce more CO2. Nanowires do that because they extend upwards, like trees. They create a forest, and you can squeeze a lot more bacteria into a three-dimensional forest than onto a two-dimensional flat surface.
TKF: And this has to take place in liquid?
P.Y.: Yes. We do this chemistry in water, where the bacteria live.
T.S.: Peidong has been a pioneer in nanowires for more than a decade. His ability to grow tall, thin nanowires is a very powerful technology that makes dense bacterial growth possible. It is the fundamental reason why this system can transfer the right number of electrons per second to the right number of bacteria.
T.M.: Catalysts, which mediate chemical reactions, generally operate more efficiently when we don't try to rush them. So the more volume these nanowires create, the more bacteria we could fit in. Then, even if each bacterial catalyst reacts slowly, you can still have a lot of output without putting in a lot of energy. And that's the whole ballgame — use less power to get more product.
TKF: I never thought of bacteria as absorbing electrons. How do they do that?
T.M.: All living things take in electrons as part of the molecules they ingest and metabolize to extract energy. We've learned now that certain bacteria can actually gather electrons through specialized thread-like structures called pili that reach out through their membranes. Those pili could play a key role in the interface between technology and biology.
Peidong, how did the electrons get into the bacteria?
P.Y.: Based on early studies, S. ovata absorb electrons directly from the nanowires, rather than through a chemical mediator. In fact, there are a host of bacteria that can do this routinely.
T.M.: Absolutely. They are just doing what life does, taking in energetic electrons, giving them to oxygen or another electron acceptor, and extracting the energy difference between these two processes to stay alive.
TKF: Did you have to genetically modify Sporomusa to do that?
P.Y.: No. S. ovata, the bacterial strain we're using, just has the amazing ability to absorb electrons and use them to process carbon dioxide into acetate.
TKF: So, what about generating fuel? Right now S. ovata transforms electrons into acetate, and E coli turns that into butanol or something else. Do you think you could do this in one step?
P.Y.: I would assume so, right, Tom?
T.M.: Sure. The ways in which we can use synthetic biology to reengineer things is almost unimaginable. Already, Pete Schultz at Scripps Research Institute has bacteria that run on 21 amino acids, one of which is completely new. The bacteria have been programed with all the genetic material and information necessary to copy this unnatural amino acid and include it as part of its metabolism. And right there at Berkeley, you've got Jay Keasling. He has bacteria that can make almost anything from acetate.
TKF: Professor Yang, could we ever make your system efficient and compact enough to use industrially?
P.Y.: In principle, it is capable of scaling up. But we would need to raise the solar-to-fuel conversion efficiency by 5 to 10 percent before we could think about commercial viability.
TKF: That conversion rate does not sound very high. How does it compare with the conversion rate of natural plants and bacteria?
P.Y.: Actually, efficiency in green plants is quite low, typically below 1 percent.
T.M.: Yes, less than 1 percent of the average annual solar energy falling on a field of crops is conserved and stored as chemical energy. That is far lower than commercially available solar cells, which produce electrical energy at 20 percent or better efficiencies, but solar cells cannot store their energy.
P.Y.: True, and by combining the best of technology and biology, we can do something similar to natural photosynthesis, but potentially at much higher efficiency.
TKF: Yes, we have talked a lot about learning from nature. Do we have the right tools to do this?
T.M.: We need all the tools we can get. We need to rededicate ourselves to basic research.
T.S.: I'm with Tom. We need more tools, and those tools come from basic science. Let me mention one that really excites me. Computational models that let us understand and predict the energetic states and reactivities of molecules, materials and catalysts.
It is a tool that brings together different researchers who frankly have a hard time talking with each other. In a room of people who study enzymes — proteins that serve at nature's catalysts — and people who research synthetic heterogeneous catalysts, the systems are so different, it can be hard to know where to start the conversation. Computational material science helps us learn from each other about how nature's catalysts differ from the ones we build artificially.
T.M.: I absolutely agree. Only a few points in a chemical reaction are actually observable experimentally, sometimes very few. Models help us understand those reactions, and how to move atoms and electrons over the low-energy pathways through these high-energy mountains. It has opened all sorts of doors already.
P.Y.: I totally agree. To come up with better synthetic catalysts, we need to learn from nature on the atomic and molecular scale. So it's very important for researchers from different research communities to come together, talk to each other, and exchange ideas.
TKF: So, what do you think you will be working on and doing in five years?
P.Y.: I think I will be trying to enhance our bacteria's efficiency and the range of chemicals they produce. More importantly, I'm very, very interested in learning how these bacteria process CO2. Hopefully, we can learn from their design and develop synthetic catalysts with decent selectivity, activity and energy efficiency .
T.S.: I don't want to repeat what Peidong just said, but I will because he is really aiming at the heart of the most important problem, learning from nature. And I'll add one additional problem that I'm really excited to study. Though we are more advanced than nature on the light harvesting side, we still have a lot to learn about how to manipulate electrons in our systems.
We also need to learn how to make light harvesting systems from materials that are not costly, toxic or energy-intensive to make. Nature synthesizes those materials at room temperature, with very low energy costs, and they use coherence effects to move energy efficiently over long distances to centers where reactions take place. I'm very excited to work on robust, biologically inspired energy transport.
T.M.: Those are profound goals. I'm not sure what I'm going to be doing in five years. I will be following what Ted and Peidong are doing, and I'm sure their discoveries will make me think about things in new ways. Out of that, I am sure I will find some new fundamental problems to work on, and I hope that work will be useful.
Follow all of the Expert Voices issues and debates — and become part of the discussion — on Facebook, Twitter and Google+. The views expressed are those of the author and do not necessarily reflect the views of the publisher. This version of the article was originally published on Live Science.
Get the world’s most fascinating discoveries delivered straight to your inbox.