World's largest gravitational wave observatory squeezes light beyond the 'quantum limit'
Researchers at the LIGO gravitational wave observatory used a new technique called frequency-dependent squeezing to boost weak signals above quantum noise.
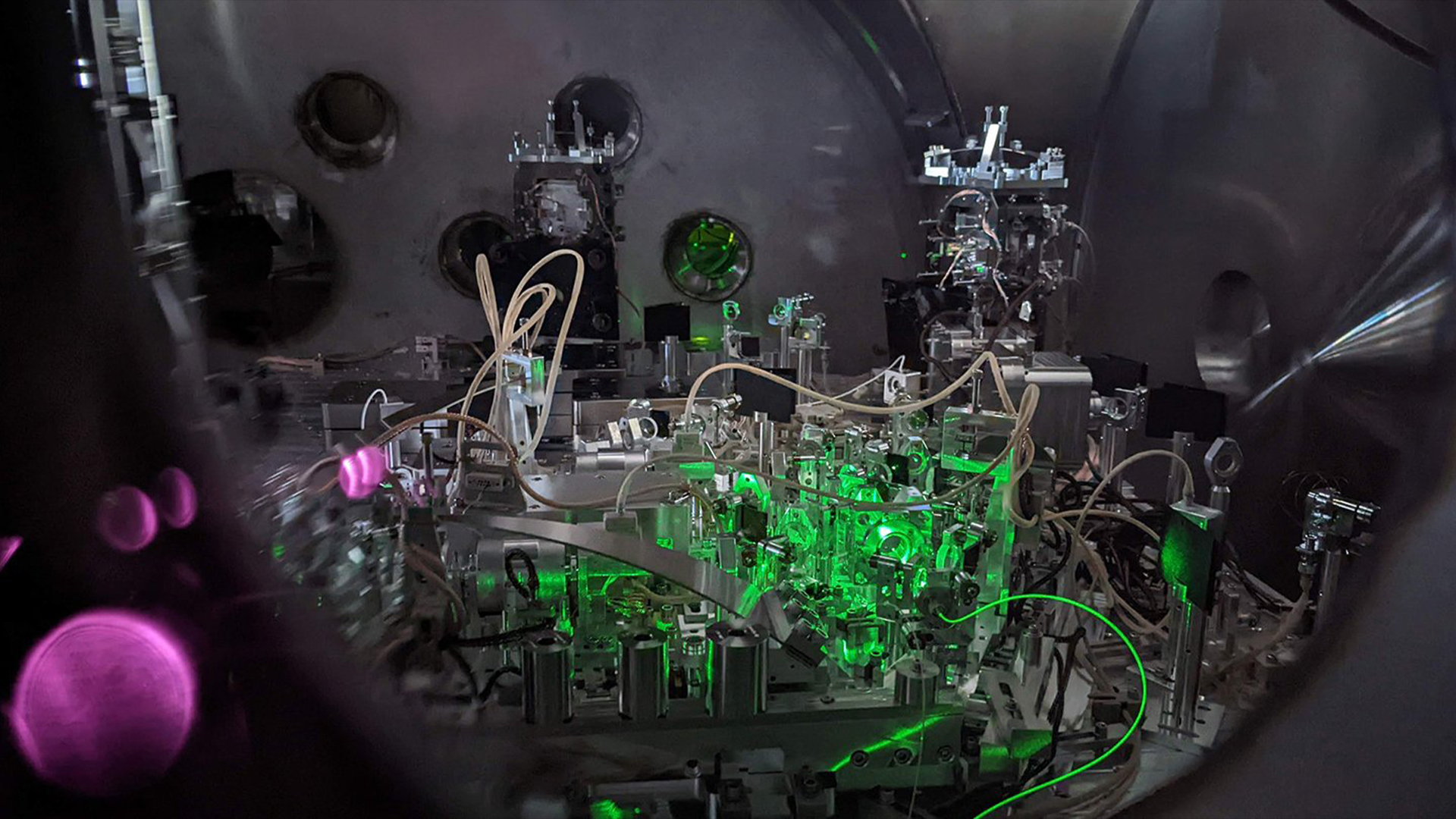
Scientists at the world's largest gravitational wave observatory have just squeezed light beyond a key quantum limit.
The new technique, called frequency-dependent squeezing, will increase the number of tiny ripples in space-time detectable by the Laser Interferometer Gravitational-Wave Observatory (LIGO), boosting the number of neutron star and black hole collisions the detector can find.
Related: Quantum 'yin-yang' shows two photons being entangled in real-time
"Now that we have surpassed this quantum limit, we can do a lot more astronomy," co-lead author Lee McCuller, an assistant professor of physics at Caltech, said in a statement.Gravitational waves ripple out when objects with mass move through space. Bigger objects — such as neutron stars or black holes — produce more prominent gravitational waves. Scientists first detected these space-time ripples in 2015 and have steadily gotten better at spotting the waves as they lap at our cosmic shores.
The LIGO detector spots these cosmic ripples from the way they distort space-time as they pass through it. Made up of two intersecting L-shaped detectors — each with two 2.48-mile-long (4 kilometers) arms and two identical laser beams inside — the experiment is designed such that if a gravitational wave passes through Earth, the laser light in one arm of the detector will get compressed while the other expands, creating a tiny change in relative path lengths of the beams arriving at the detector.
But because these distortions are so tiny — often the size of a few thousandths of a proton or neutron — LIGO's detectors must be incredibly sensitive. So sensitive, in fact, that their most precise measurements are muddied by noise from quantum effects, or the spontaneous interactions of subatomic particles.
High frequency noise comes from tiny particles randomly popping in and out of existence. Low frequency noise comes from the rumble of reflecting light particles that cause the mirrors to wobble. Both sources limit the number and types of gravitational waves LIGO can detect.
Get the world’s most fascinating discoveries delivered straight to your inbox.
To break through these quantum limitations, the physicists turned to another principle of physics: Heisenberg's uncertainty principle, which states that we can only simultaneously know specific pairs of a particle's physical properties to a set level of certainty.
This means that there is a trade-off in how well scientists can measure both the amplitude (or power) and frequency of the light inside LIGO, but it also means that either property can be amplified at the cost of the other. By using crystals that split individual photons, or packets of light, into two entangled photons, the physicists tuned the light so that the uncertainty behind its amplitude or its frequency could be "'squeezed"' as required.
Frequency-dependent squeezing works a bit like pinching a balloon, the researchers say. Just as pinching a balloon at one end helps the other end get bigger, pinching one property of light to know it with greater certainty moves the overall uncertainty to the other. This means that at low frequencies, a squeezed amplitude reduces noise from the mirror rumbling, and at high frequencies a squished phase makes the signal stronger than noise from quantum perturbations.
"It is true that we are doing this really cool quantum thing, but the real reason for this is that it's the simplest way to improve LIGO's sensitivity," co-lead author Dhruva Ganapathy, a graduate student at MIT, said in the statement. "Otherwise, we would have to turn up the laser, which has its own problems, or we would have to greatly increase the sizes of the mirrors, which would be expensive."
The findings were published Sept. 6 in the journal Physical Review X.
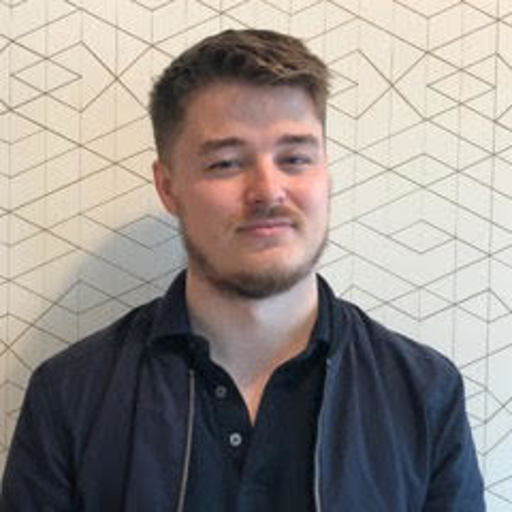
Ben Turner is a U.K. based staff writer at Live Science. He covers physics and astronomy, among other topics like tech and climate change. He graduated from University College London with a degree in particle physics before training as a journalist. When he's not writing, Ben enjoys reading literature, playing the guitar and embarrassing himself with chess.