Hunt for Why We Exist Turns to Weird Atomic Decay
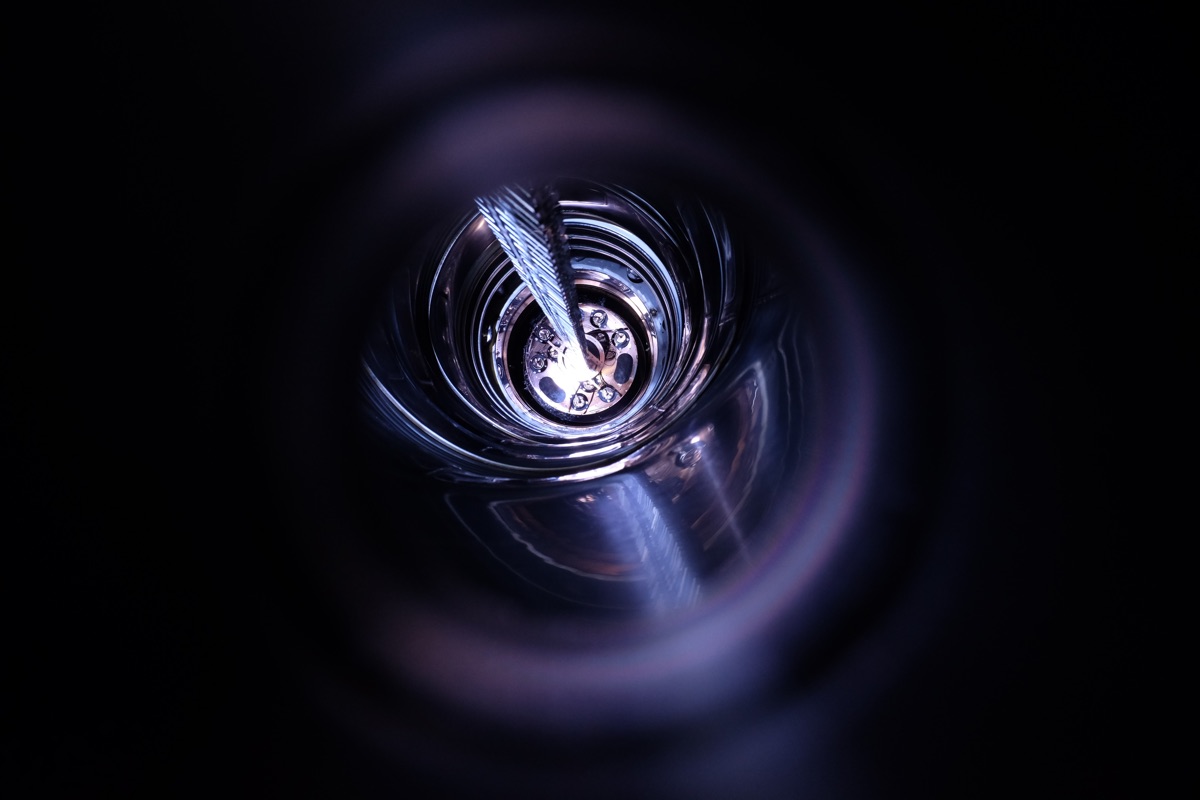
A never-before-seen type of radioactivity could explain why matter, including humans, exists today. And now a team of physicists has launched an experiment to find the oddball phenomenon.
When the universe first formed some 13.7 billion years ago, current theories say that equal amounts of matter and its bizarre cousin, antimatter, should have been produced during the Big Bang. Physicists know that when the two come into contact, they annihilate each other — poof. If that were the case, though, nothing should exist except photons and neutrinos. And yet, here we are. Calculations show there was a tiny bit more matter than antimatter — enough so that things exist — but why?
One way to explain this matter-antimatter asymmetry is to look for some difference between the two, besides charge, that could explain matter's advantage. It's a big question in contemporary physics, because otherwise matter and antimatter should, per contemporary theories, behave the same way. [The 18 Biggest Unsolved Mysteries in Physics]
Weird neutrinos
In this new study, physicists are seeking so-called neutrinoless double-beta decay. Normally, some radioactive atoms' unstable nuclei will lose a neutron via beta decay — the neutron transforms into a proton by releasing an electron and a tiny particle called an electron antineutrino. A mirror image can also occur, in which a proton turns into a neutron, releasing a positron and an electron neutrino — the normal-matter counterpart to the antineutrino. Double-beta decay happens when two electrons and two antineutrinos (the antimatter counterparts of neutrinos) are released: basically, the beta decay happens twice. Scientists have long theorized a neutrinoless version of this process — something that would suggest that the two neutrinos annihilated each other before being released from the atom. Essentially, the neutrino behaves as its own antimatter sibling.
(Particles of matter that are their own antiparticles are called Majorana fermions, after the Italian physicist Ettore Majorana, who hypothesized their existence in 1937.)
If neutrinos and antineutrinos behave differently from each other, that could help to explain why all matter wasn't annihilated at the instant the universe formed. [5 Elusive Particles That May Lurk in the Universe]
Detecting decay
Finding this odd event though, is hard to do, because there's so much background "noise," said Bernhard Schwingenheuer, the spokesman for the GERmanium Detector Array (GERDA) collaboration. The background "noise" comes largely from cosmic rays.
Get the world’s most fascinating discoveries delivered straight to your inbox.
So the physicists turned to GERDA. Nestled in an underground lab in Italy, the GERDA experiment consists of detectors in a bath of liquid argon that is enriched with the isotope germanium-76, which is mildly radioactive. It has a half-life of 1.78 x 1021 years (or 1.78 billion trillion years) — meaning that it takes that amount of time for half of its atoms to turn into selenium, which is many orders of magnitude longer than the universe's 14-billion-year age.
Usually, germanium will emit two electrons and two electron antineutrinos — the ordinary double-beta process — when it transforms via its slow decay. The physicists wanted to see if this ever happens without releasing the neutrinos: the long-sought neutrinoless double-beta decay.
With such a long half-life, one might think it would take too long to see this happen, but a half-life is a probabilistic phenomenon. This is why the experimenters used some 84 lbs. of germanium mixed with the liquid argon: that yields about 4.5 x 1025 (or 45 trillion trillion) atoms, which means at least a few of them should undergo the decay while scientists are watching.
The GERDA team gathered data for about seven months, from December 2015 until June 2016. They didn't find the decay, but they could put a lower limit on how often the decay happens: It has a half-life of 5.3 x 1025 years, which means you'd have a 50-50 chance of seeing a single atom do it in that amount of time.
Extending the Standard Model
If they find it, that would mean that neutrinos are their own antiparticle, like photons — the neutrinoless decay couldn't happen unless that were the case. It also means that this kind of radioactive decay is not symmetrical. Recall that beta decay has a mirror image — either electrons and antineutrinos or positrons and neutrinos are emitted. If the double-beta decay isn't symmetrical, that means that neutrinos and antineutrinos behave differently. This isn't true of other kinds of particle-antiparticle pairs, as far as anyone knows.
This phenomenon would affect the Standard Model, which has been a wildly successful way to describe particle physics, but it's clearly incomplete. The model predicted the existence of the Higgs boson particle. However, Schwingenheuer noted that there is evidence that neutrinos have a tiny mass (only discovered in 1998, which won the Nobel in 2015) and that dark matter exists — indicating that the Standard Model isn't the last word.
"If neutrinoless double-beta decay is observed, it helps solve a couple of problems," said Philip Barbeau, an assistant professor of physics at Duke University in an email to Live Science. "For one, it helps to explain the matter-antimatter asymmetry in the universe. It also helps explain why neutrino masses are so surprisingly small. We would also get an idea of the neutrino masses as well, as the decay rate is related to the mass scale of the neutrinos."
The question then becomes what kind of physics lies beyond it. The GERDA experiment has yet to reveal the decay that the researchers seek, but that doesn't mean it won't in the future, Schwingenheuer said. And it would be difficult to rule it out entirely, because it's possible that the timescale is just longer than they think. Right now, they've set a lower limit on the half-life for this decay, but further experimental runs could push that number up.
As for what happens if they don't see the odd decay after many runs, Barbeau said it might not be a deal breaker for new models. "We don't go back to the drawing board from the point of view of the underlying theories. We just won't know whether neutrinos are Majorana or not."
The research is detailed in the April 6 issue of the journal Nature.
Original article on Live Science.
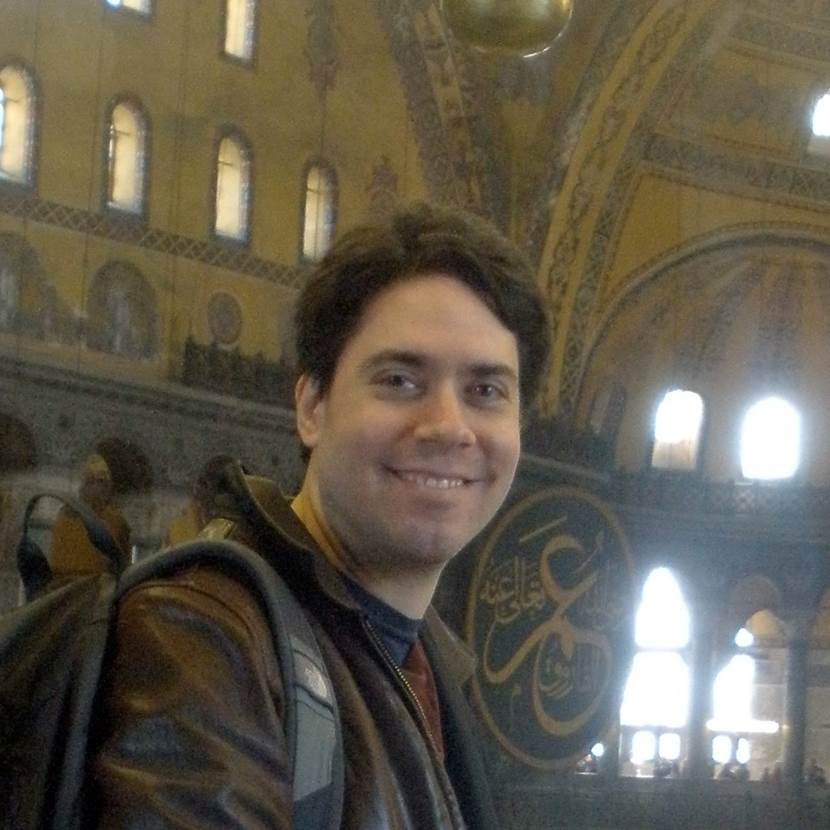