Book Excerpt: 'The Tide: The Science and Stories Behind the Greatest Force on Earth'
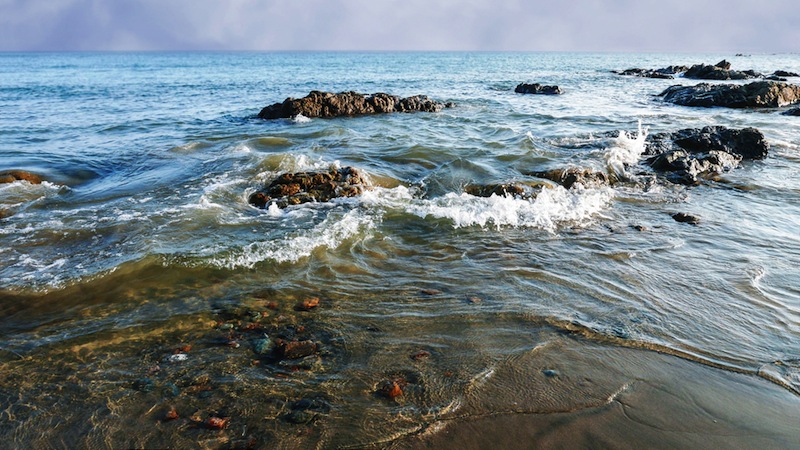
A rich and sweeping exploration into the science and history behind the most mysterious, primal, and powerful force on earth: the tide. Half of the world's population today lives in coastal regions lapped by tidal waters. But the tide rises and falls according to rules that are a mystery to almost all of us. In "The Tide," celebrated science writer Hugh Aldersey-Williams weaves together centuries of scientific thinking with the literature and folklore the tide has inspired to explain the power and workings of this most remarkable force. Below is an excerpt from Aldersey-Williams' "The Tide" (W. W. Norton & Company, 2016).
Tide Sense
How do animals "know" what the tide will do? When scientists began to study them, they thought it unlikely that grunions, for example, could have a wholly internal mechanism by which to time their breeding run. Do they then sense the tide directly, or are they responding to other stimuli? Certain things can be deduced. It cannot be moonlight that causes the fish to run ashore, as they do this at high-water springs both on the bright full moon and in the darkness of the new moon. The fact that spawning occurs not on the very highest tides, but shortly afterward, indicates that the behavior is not directly governed by tide height either (something that the grunion might detect as increased water pressure around its body). It has recently been found that it is agitation in the sea when the next spring tide comes that stimulates the grunion eggs to release their larvae by generating enzymes that dissolve the egg membrane. But wave action cannot be the trigger for the original beaching, or else storms would disrupt the pattern of the spawning runs.
What else is left? Could the stimulus be pure gravitational attraction? Any forces felt by the fish would be minute in comparison with the changes in pressure that they tolerate when swimming at different depths, but gravity cannot be entirely discounted. Whatever triggers the behavior, it seems it is not confined to the single tide when the grunions come ashore to reproduce, since the eggs begin to mature long before they are spawned—and this, too, has been found to happen in concert with moon phases.
The author John Steinbeck writes about this conundrum in The Log from the Sea of Cortez, the record of a specimen-gathering expedition he made to the Gulf of California with his friend Ed Ricketts in 1940. (The Sea of Cortez was the name of his vessel as well as his happy hunting ground.) Ricketts—who provided Steinbeck with the model for the character of Doc in Cannery Row—later wrote a scientific guide to intertidal life based in part on what the two men collected on the trip. Tangling with mangrove roots in their motor launch at high water, and racing against time to gather specimens from the uncovered beds at low tide (like knots), they find an astounding diversity of colorful life in the warm waters: crabs and snails, and creatures with names out of horrible myth, such as the gorgonian, and others, like the serpulids, so obscure that their only name is the exotic-sounding one given to them by science. They see fish that can survive out of water, at least for the period of one tide, and minutely observe how the foreshore is graded with different kinds of life by height and by the time spent immersed in seawater.
Inevitably, they find themselves drawn into speculation on the importance of the tide to this abundance of life, especially since, in Precambrian times, when single-celled organisms began to evolve into more complex forms in the sea, the tides were far greater than they are today, because of the closer orbit of the moon. Steinbeck writes, "The moon-pull must have been the most important single environmental factor of littoral animals." Their body weight and displacement in the sea would have cycled strongly with the rotation of the earth. "Consider, then, the effect of a decrease in pressure on gonads turgid with eggs or sperm, already almost bursting and awaiting the slight extra pull to discharge." What Steinbeck finds more remarkable is that so many creatures seem to have carried forward a kind of ancestral memory of this response and fine-tuned it to the far weaker signal of tides now—an effect to which, he believes, even we are not immune. "Tidal effects are mysterious and dark in the soul, and it may well be noted that even today the effect of the tides is more valid and strong and widespread than is generally supposed."
But we are still left with the question of how it is that these creatures respond to the tides. They do not go around with tide tables; they do not superfluously relate tide to time as we do. So either they must have some kind of built-in tidal clock, or they must directly sense some primary or secondary property of the tide, which might include the pressure or rate of flow of water, or its change in temperature or salinity.
Perhaps our own removal from the tides is making the problem seem harder than it is. It is, after all, no miracle that animals are sensitive to time. We ourselves are slaves to the circadian rhythm, as our alarm clocks and news bulletins daily remind us. Why should a circatidal rhythm be any odder than a circadian one? Of course, the circadian rhythm has the obvious cues of bright sunlight alternating with utter darkness. But this is what is obvious to us. What cues might be just as obvious to marine creatures very different from ourselves? We find a twenty-four-hour cycle perfectly natural, but might not these animals find a period of twelve-and-a-bit hours just as natural, especially if their survival depends on it?
Get the world’s most fascinating discoveries delivered straight to your inbox.
. . .
More recent research has revealed even stranger ways in which marine creatures harness the tide. A Pacific species of starfish, for example, the purple sea star, Pisaster ochraceus, has developed a water-cooling system to help it survive accidents of tidal stranding. This curious response is triggered when the animal first becomes hot as the sea leaves it behind on one low tide. This unhappy event triggers a mechanism that enables the sea star to retain some seawater when the tide next rises and then recirculate it when the tide falls once more so that it does not experience the same discomfort again.
Where survival is not at stake, the drive is often to conserve energy—the explanation of so many natural adaptations. There is an equivalence between food and energy: food provides energy, but it costs energy to find food. If the tide brings in a food supply, then that represents a saving of energy, like having groceries delivered to your door. For any sea creature, the tides are a huge source of free energy there for the taking.
This is why places where the tides are large or fast-running are often high in biodiversity. Some creatures draw an energy advantage from the movement of so much water, while others are drawn in to feed on them, building a rich ecosystem. This richness, in turn, is a large part of what gives tidal locations their strong sense of place. These places are usually on or close to the shore, but they may also occur over undersea ridges or shelves where the tide runs in such a way as to mix waters from different depths, churning nutrients and dissolving oxygen from the air. It is striking that the larvae of the eel, whose life cycle had greatly baffled naturalists since Aristotle because they could not determine the sex of the creatures and had never seen their young, were originally detected in the Strait of Messina as late as 1856, although they were at first misidentified as a new species of fish. It seemed that the mysterious larvae were drawn up from the depths by the turbulent Charybdis whirlpool, and it was presumed that the eels spawned nearby in unknown depths. It was another fifty years before the Danish biologist Johannes Schmidt established the vital importance of the Sargasso Sea in the life cycle of the eel, and showed that the Mediterranean was not a breeding ground. The young fish were perhaps drawn to the Messina Strait for its rich food supply.
The flattened variety of sea urchins known as sand dollars may be adapted to take advantage of the tide in another way. Their shells, or tests, exhibit the usual fivefold symmetry of this class of echinoderms, but they are also often pierced with elongated holes, called lunules. One species, which rejoices in the name Mellita quinquiesperforata, has five of these holes, but others may have different numbers, and in different places on the shell, not governed by the overall fivefold symmetry. The variable shape and number of these features poses a puzzle to zoologists. The lunules do not assist with feeding or offer any structural advantage to the test. In 1981, however, Malcolm Telford, a marine biologist at the University of Miami, compared the way water flowed around a perforated and a nonperforated species in a water tank, and found that when the water was flowing fast enough, the perforated sand dollar was able to channel the flow through its shell in such a way as to generate lift and stabilize its vertical displacement in the water. The lift occurs in water moving at speeds typical of tides in shallow waters, suggesting that the holes might have evolved as an energy-saving strategy, enabling the animals to ascend or descend in the running tide with no more than a slight tilt of the body.
Other marine species use the tide to hitch a ride even more blatantly. Julian Metcalfe is one of a group of scientists at the UK Centre for Environment, Fisheries and Aquaculture Science (CEFAS) interested in fish migration and its implications for the sustainable management of commercial fish stocks. The government organization is based in Lowestoft, formerly one of the biggest fishing ports on England's North Sea coast. It occupies a suitably forlorn complex of brick buildings that began life as a hotel when vacationers were still satisfied with sea views painted entirely in shades of gray. If one wanted a satirically British counterpart to the sun-soaked modernism of the Pacific-facing Scripps Institution, one could hardly do better.
One focus of Julian's research is the plaice, one of the large edible flatfish traditionally landed here. Like many species of flatfish, plaice are known to lift themselves into the tidal stream when it is running in the direction they wish to go and to drop out to lie on the seabed when the flow is unfavorable. The behavior contrasts with bigger, round fish such as cod and tuna, which are unable to burrow into the seabed to escape the tidal currents, and so usually find it more advantageous just to keep swimming. The plaice are perfectly capable of swimming too—and they do so where the waters are not strongly tidal—but they prefer to save energy where they can.
By tracking fish using imaging sonar from the CEFAS research vessel, and more recently by collecting data from electronic tags fitted to individual fish, Julian and his colleagues have been able to map the shifting populations of the fish through the seasons. It emerges that they are adept users of the tide. When the plaice are spawned, the eggs and tiny larvae of the fish are first carried helplessly by tidal currents into shallow coastal nurseries, where they are able to grow at reduced risk of predation. Fish spawned off the Thames estuary, for example, may be transported as new-hatched eggs toward the Frisian Islands off the Dutch coast. There the tide whisks them through the Texel island gate between two of the islands into the shallow and protected Waddenzee, where they grow up to 20 centimeters long. The young fish are able to stay safely within the nurseries by resting on the seabed when the tide is ebbing, and rising up into the water when it is flooding. They do this using a kind of internal tidal clock that is sensitive—or "entrained," in the jargon of the field—to changes in water pressure produced by the changing height of the tide.
When they have grown to adulthood, the plaice are able to migrate between their winter spawning grounds in the North Sea and their ocean feeding grounds, riding on the tide when it is running in a favorable direction and dropping to the bottom when it is not. They are thought to use a combination of both circatidal and circadian biological clocks to do this. The plaice can tell whether a tide is running to a high degree of accuracy, remaining on the seabed during slack water, but lifting off within minutes of its beginning to run their way. But there remain unanswered questions about this behavior: How do the fish know where to go? How do the fish sense the direction of the tide? And how, with no change in tactile or inertial sensation, do they know when the free ride is over and the tide that has been bearing them along comes to a halt, especially if it is dark and there are no visual cues?
For Julian, the plaice is a highly convenient fish to study. The technology and resources were made available for research because it is a commercially important species; plaice is third in the trio of popular fish-and-chip-shop varieties of bottom-living fish caught in the United Kingdom, after cod and haddock. But the animal has since been shown to exhibit a migratory behavior that is fascinating in its own right. Julian is convinced that similar mechanisms exist in many species. "I can't believe lots of animals don’t take account of water currents," he says. "We can now modify our environment so much, we forget the old cues, the ones that animals still use."
Solar control of the daily and yearly rhythms of life on land is relatively well understood (and of course inescapably familiar in ordinary human experience). But an explanation of the rhythmic mechanisms of marine life is only just beginning to emerge. Circadian rhythms are regulated by genes that provide chemical feedback. This means that the rhythm is maintained in a "free-running" fashion even in the absence of external stimuli such as changing light levels or temperatures. Similar free-running rhythms are seen in sea creatures, but it has been arguable whether these biological clocks are truly tide related or are versions of the circadian clock tweaked by the processes of natural adaptation to operate at a different rate.
In 2013, however, geneticists at the University of Leicester obtained evidence to suggest that a dedicated circatidal biological clock does exist. Researchers led by Charalambos Kyriacou worked with the speckled sea louse, a familiar denizen of intertidal sandy beaches that bears the deceptively alluring Linnaean name Eurydice pulchra. By disrupting the expression of genes responsible for circadian timekeeping, and showing that the animals nevertheless maintained their normal tidal behavior, they established that the tidal rhythms are driven independently by a circatidal clock. The lice have biological clocks of both kinds: a circadian clock that governs such matters as the production of pigments in the body, and the circatidal clock that regulates their swimming activity in response to the twelve-hourly cycle between successive high tides.
Perhaps many creatures have the potential to switch over to circatidal rhythm. On the walled island of North Ronaldsay, the northernmost of the Orkneys, the local sheep were excluded from grazing land in 1830 when the laird decided to use the lush grass to breed cattle. Except in the lambing season, the sheep are confined to the beach side of the coastal wall. Over the years, the animals have adapted to a diet of seaweed and a grazing timetable governed by the tides that uncover it.
As for the longer cycle of spring and neap tides (the word "circalunar" is used to distinguish this period from the circatidal response to each tide), there is new evidence to explain animal responses to this too, from similar experiments at the Max F. Perutz Laboratories of the University of Vienna. The Austrian researchers used rag worms for their subjects. This was one of the first species observed to spawn on a cycle attuned to the spring tides and is considered a living fossil, with a physiology, behavior, and habitat unchanged over millions of years. Unlike the grunion, it does not spawn on every spring tide at the right time of year, but only monthly, on the spring tides at the new moon. This behavior suggests that the animal's circalunar clock may be entrained to moonlight, or rather the absence of moonlight, and not to the hydrodynamic factors that might be important for the grunion. Biochemical reactions catalyzed by lunar light may play a role in this programming.
All known biological clocks are linked ultimately either to the sun or to the moon. The discovery of circalunar clocks in animals must surely raise the hopes of those many horticulturists and farmers who believe, scientifically or not, that their seeds are better planted and their crops are better harvested at certain phases of the moon. And what about us? The wife-and-husband team who led the Viennese research, Kristin Tessmar-Raible and Florian Raible, conclude their paper with this bold question: "Is it possibly more than sheer coincidence that the female reproductive cycle in humans lasts around a lunar month, or could this instead reflect some regulatory left-over from our evolutionary past?"
I had, of course, been entirely unaware of the dependence of the rag worm on the lunar cycle when I picked one out of the mud during my day observing the tidal cycle on the north Norfolk coast. I saw gulls and curlews come and go that day too, but I would have had to stay for many more days in order to appreciate all these animals' obeisance to the tides. It is surely a mark of how much our own lives are ruled by the black and white of day and night that we are so insensitive to these different rhythms, and it is only now that science is beginning to comprehend them.
Pooling Our Knowledge
With this abundant evidence that life depends in many ways upon the tides, we are entitled to ask whether life could have evolved on earth at all in their absence.
Charles Darwin has little to say about the tides in On the Origin of Species. He uses the word only three times—twice in a metaphor, and once in a geological argument in relation to the erosion of cliff bases. Yet by the time the book was published in 1859, and possibly for some time before that, he firmly believed that life must have had an aquatic origin. However, he forbore speculating on the matter in print, publicly saying it was "rubbish" to think of doing so, and it was only much later, in a short letter to the botanist Joseph Hooker in 1871, that he made the remark that soon became famous, imagining the "warm little pond" where the right simple chemicals might first have converged:
It is often said that all the conditions for the first production of a living organism are now present, which could ever have been present. But if (& oh what a big if) we could conceive some warm little pond, with all sorts of ammonia & phosphoric salts,—light, heat, electricity, &c, present, that a protein compound was chemically formed, ready to undergo still more complex changes, at the present day such matter wd be instantly devoured, or absorbed, which would not have been the case before living creatures were formed.
The scenario soon captured the public imagination. In The Mikado, Gilbert and Sullivan’s comic opera of 1885, Pooh-Bah claims descent from "a protoplasmic primordial atomic globule."
Darwin presents in a nutshell the conundrum that still baffles scientists today. For the continuing existence of life may paradoxically be the very thing that prevents us from observing how it emerged in the first place. The fact that life is not observed spontaneously forming on the present-day earth leaves the question of its origin wide open.
The immediate context of Darwin's letter to Hooker was the fact that Louis Pasteur had shown by experiment that it was impossible to generate germs spontaneously, without there first being some other organic matter present. Darwin never expressed a view on how the first germ came about. Among the theories seriously entertained by others were and are that the necessary chemicals were delivered to this planet by comet impacts or, alternatively, that they were thrust up to the surface of the earth from deep within its crust. Several reputable scientists, including Darwin's champion Thomas Huxley, even made excited claims to have found protoplasm in mud from the bottom of the sea.
For all Darwin’s stature, a "warm little pond" is not currently regarded as the most likely stage for the emergence of life. It has been superseded—for the moment at least—by hydrothermal vents on the seabed. These vents eject heated, mineral-rich water into the deep ocean like hot springs on land. Here, hot gases and simple organic molecules might have mixed and combined in darkness to synthesize the first amino acids and other precursor compounds of life. The darkness is believed to be important because strong ultraviolet light from the sun is known to break up such delicate molecules. The Archaean ocean that covered most of the earth four billion years ago was rich in iron and other metals that might have catalyzed these early reactions, doing the job now done by the biological molecules called enzymes.
Nevertheless, the pull of the moon remains strong, and it is still easy to find scientists who cling to the idea of an origin somewhere on the tidal shores of that great ocean. Even dry textbooks occasionally betray this sentiment. David Pugh and Philip Woodworth's 2014 Sea-Level Science is one. "It is highly likely that we would not be here without the Moon and the tide," they write.
Part of the reason for this favoritism may lie with our deep psychology and the fact that water is central in so many creation myths. But it is one thing to say that life emerged on the edges of the sea, quite another to say that it was necessary for that sea to be tidal or that there had to be a moon in the sky.
In 1952, a graduate chemist named Stanley Miller and his supervising professor, Harold Urey, at the University of Chicago designed an experiment to simulate Darwin's pond. They combined a mixture of ammonia, methane, hydrogen, and water vapor—the obvious gaseous ingredients of amino acids—and provided an energetic stimulus in the form of heat and an electrical discharge in imitation of the lightning thought to have been a feature of the violent weather on the early earth. After a day of this battering, the water in their flask turned pink. By the end of the week, it was red and turbid with organic compounds, including three of the simplest amino acids.
Miller and Urey may have made "primordial soup" in 1952, but they did not consider the soup bowl. Was the crucible for this reaction supposed to be the atmosphere (they vaporized the water and mixed it with the other gases) or the turbulent ocean (the amino acids were found afterward in the liquid condensate)? In the latter case, shouldn’t the soup have been salty? Many say our earliest environment must have been rich in salts because the organisms that evolved from it can tolerate and often require high levels of salts. Yet Miller and Urey's experiment did not include the salts and minerals known to be in the sea. Might it then have been in calm tide pools warmed by the sun that these essential chemicals were able first to concentrate before being decanted at regular intervals into the protective vastness of the ocean?
Before we get carried away with this cozy picture, it is necessary to recall that the tides when the molecular precursors of life developed were very different from those we experience today. When the earthmoon system was formed around 4.5 billion years ago, the moon was held at first in the closest orbit it could be in without collapsing into the earth only a few thousand kilometers away. A little later—that is to say, a few hundred million years later—when the earth's water first condensed into rains that themselves must have lasted for millions of years, the moon’s orbit was still extremely close. The first tides would have been enormous and violent. It is in this challenging environment, riven not only by these tides, but also by volcanic eruptions and savage storms, that the first amino acids and then the complex proteins must have formed.
Aside from raising huge tides, the strong gravitational field of the moon served to stabilize the earth on its axis, which was an important factor in making life sustainable by limiting extremes of climate.
Even four or five hundred million years ago, long after the Cambrian explosion of life on earth, the moon was still significantly nearer than it is today and the tides still very high. With more than four hundred days in the year, each day was a little shorter than it is now, leaving these big tides less time to ebb and flow, so giving rise to powerful currents.
The scale of these early tides can be tentatively estimated by examining ancient rocks called tidalites, or tidal rhythmites, in which sediment layers have been built up and shaped by the regular ebb and flow of the water, although such features are thin on the ground, since they must have been raised above sea level by subsequent geological events in order not to be covered or eroded by the action of later tides. The oldest known deposit, created by tides around 750 million years ago, lies in Big Cottonwood Canyon, Utah, in which the varying force of the tides is indicated by alternating light-colored sandy layers left by strong tides able to transport sand and darker layers of mud deposited by weaker tides. The tides in this Precambrian Era may have had a range of 50 meters or more and would have run in and out at huge speeds. Beach vacations would have been challenging, but the tide pools very numerous and lively.
After his headline-grabbing synthesis of amino acids, Stanley Miller unsurprisingly made the chemistry of prebiological molecules his life's work. Scientists' ideas concerning the mix of gases and minerals available for this synthesis changed many times, but each time, Miller was able to create amino acids and other simple organic compounds. This is the easy part. Even if Miller's experiments were faithful simulations of what happened billions of years ago, nobody has been able to replicate the equally vital later stages of the process, knitting together those amino acids and other compounds to make proteins, RNA, and DNA. If anything, though, it is more likely that it is these later, more complex syntheses that require tide pools for their success. In a paper published in 1990, Miller and his coauthors speculated that DNA may first have been encapsulated in the protective fatty layers called liposomes in the intertidal zone, as also happens today. Even if this was the case, however, there is no explanation yet of how DNA was made in the first place. Was this stage, as well as the preceding one of building the proteins, accomplished in tide pools?
The answers to these questions will not only inform our knowledge of how life on earth began. They may also narrow the search for life on other planets. If a moon large enough to raise significant tides is necessary for evolution to take place—in addition to oceans and the right mix of chemicals—then the number of candidate planets we should be looking at will be greatly reduced.
Copyright © 2016 by Hugh Aldersey-Williams. Used by permission of W. W. Norton & Company, Inc. All rights reserved.